by
Jimmy Marty
Establishing accurate sediment core chronologies is of great
importance for the interpretation of paleolimnological records. Radiocarbon
dating is the primary method used to create chronologies in studies focusing on
the past 40-50,000 years. Described here are various macroscopic plant
materials, categorized according to their suitability for radiocarbon dating. Materials
were evaluated based on the characteristics of good dating material: a)
all fixed CO
2 is in equilibrium with atmospheric CO
2 and
b) the material accurately represents the time of sediment deposition.
Sources of error
A common age-altering error encountered in paleolimnology is
the old-carbon error, which occurs when dating material has fixed carbon out of
equilibrium with the atmosphere. Old carbon reservoirs are commonly associated
with hard-water lakes with high concentrations of HCO3-,
but sediment and aquatic CO2 are often old-carbon enriched due to isotopic
exchange between carbon species (Mook 1980), infrequent mixing (in lakes with
high surface area-depth ratios) (Hakkanson 1979; Olsson 2009), and respired CO2 derived
from HCO3- fixing organisms (MacDonald et al 1987). This list does not include
processes in arctic and volcanic regions, which may be old-CO2 enriched for
numerous other reasons (see Bjorck and Wohlfarth 2002).
Physical processes such as reworking of old sediment by
means of erosion or bioturbation can redistribute plant remains up or down the
sediment profile (Turney et al 2000; Bjorck and Wohlfarth 2002). Sediment disturbance
is often clear through visual examination of the sediment core face, in which
slumps and/or turbation may be evident. Further examination of microscopic
composition using smear slides can reveal less obvious terrestrial material
indicative of erosional input. Describing these macroscopic and microscopic
features of the sediment is of great value to the selection of suitable
emergent and floating leaved macrofossils and can help avoid erroneous dates.
Appropriate sample storage techniques should always be
performed in order to avoid contamination errors. Sediment cores and macrofossil samples stored in cool and wet conditions for several months or more are susceptible to modern fungal and microbial contamination (Wohlfarth et al 1998). After isolating the macrofossil sample from the sediment, it should be stored in distilled water in a cold room for no longer than one week before submission for dating (Bjorck and Wohlfarth 2002).
Dateable: (click on heading for more comprehensive list of taxa in this category)
Terrestrial plant
macrofossils
Terrestrial plants fix only atmospheric CO2 and are
considered ideal for radiocarbon dating. Care should still be taken to ensure
the material has not been reworked through sediments.
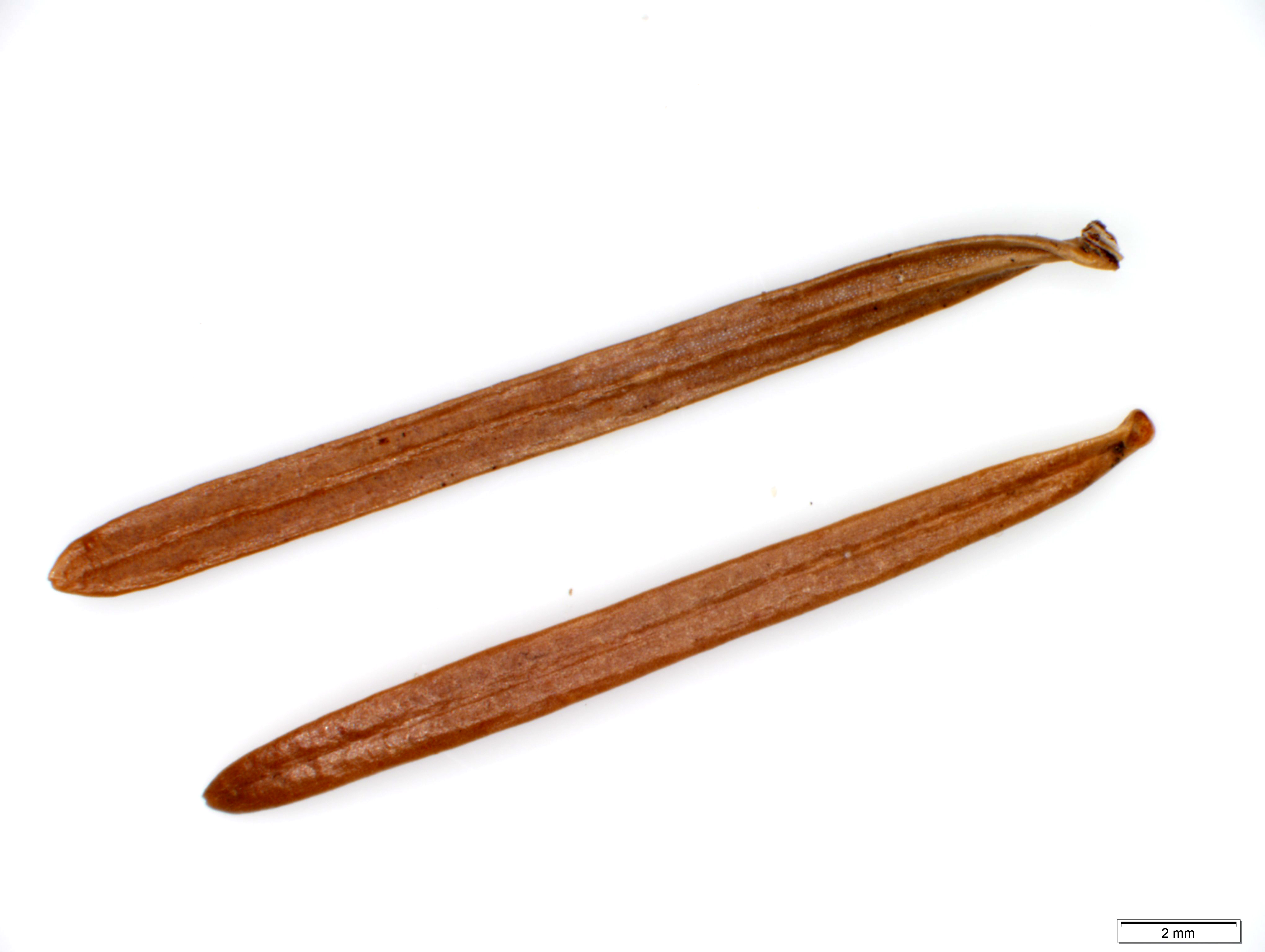 |
Pine needle (terrestrial) of Abies balsamea. Dateable. |
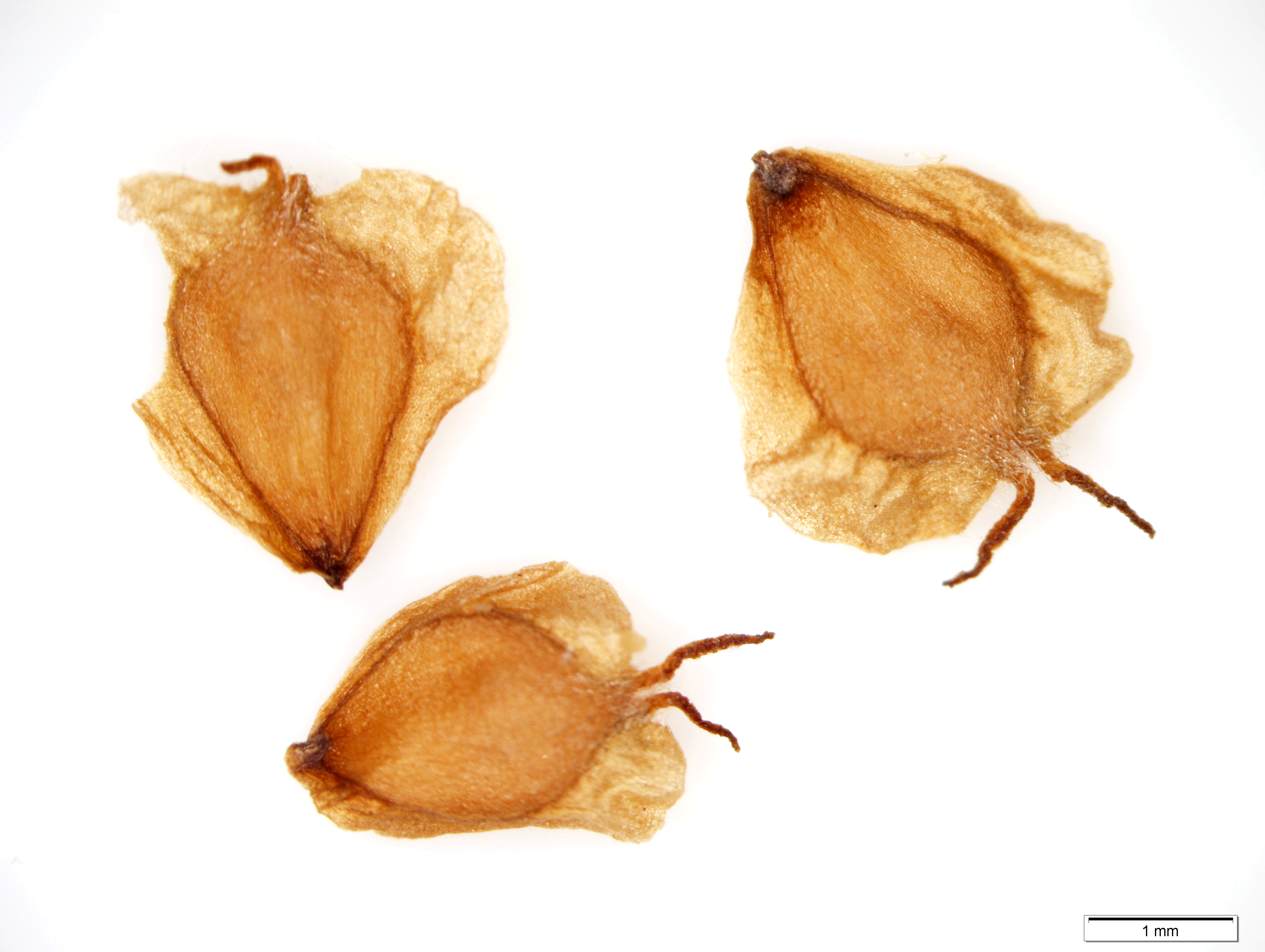 |
Seeds of terrestrial tree Betula alleghaniensis. Dateable. |
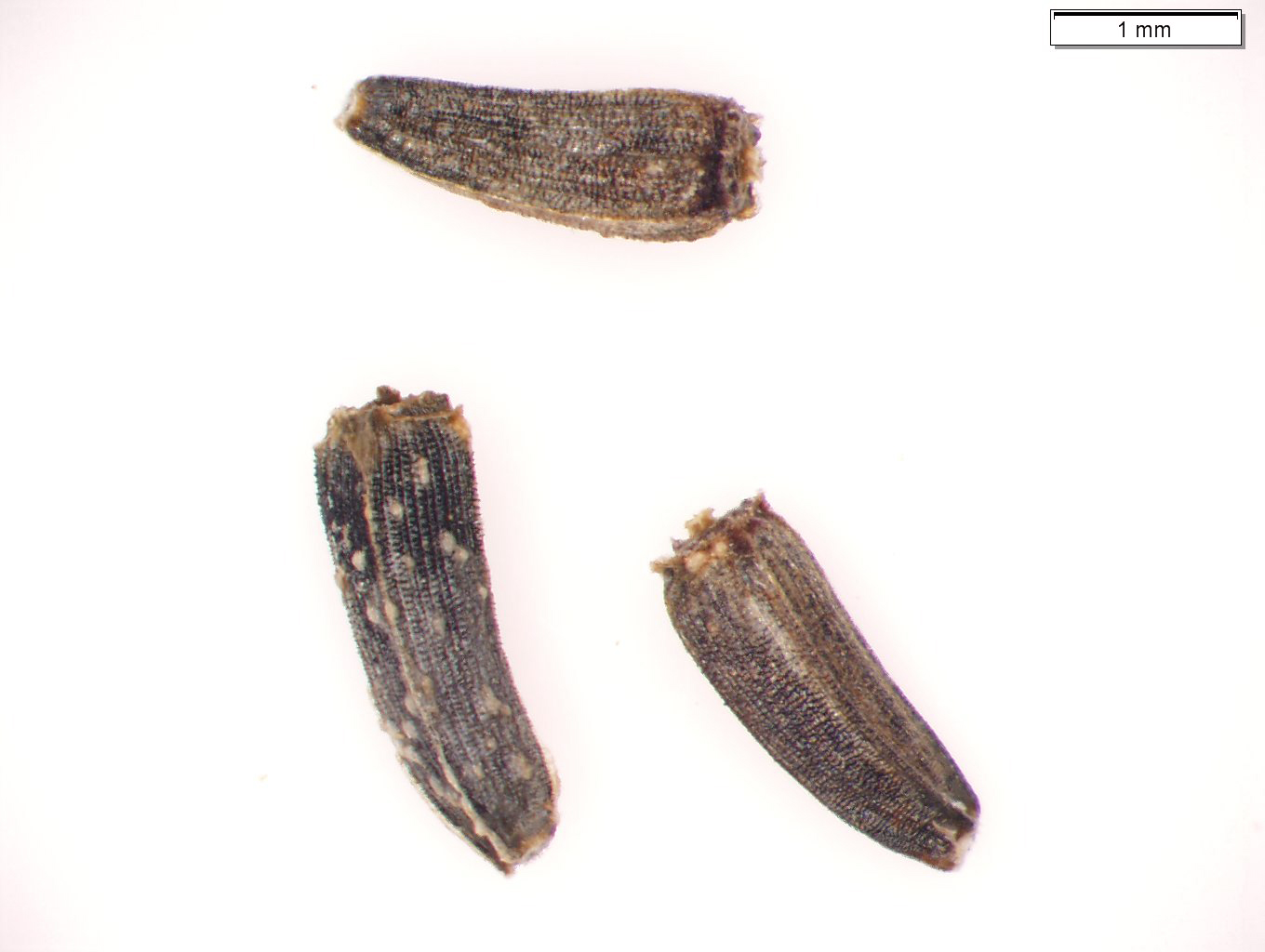 |
Seeds of terrestrial herb Rudbeckia triloba. Dateable. |
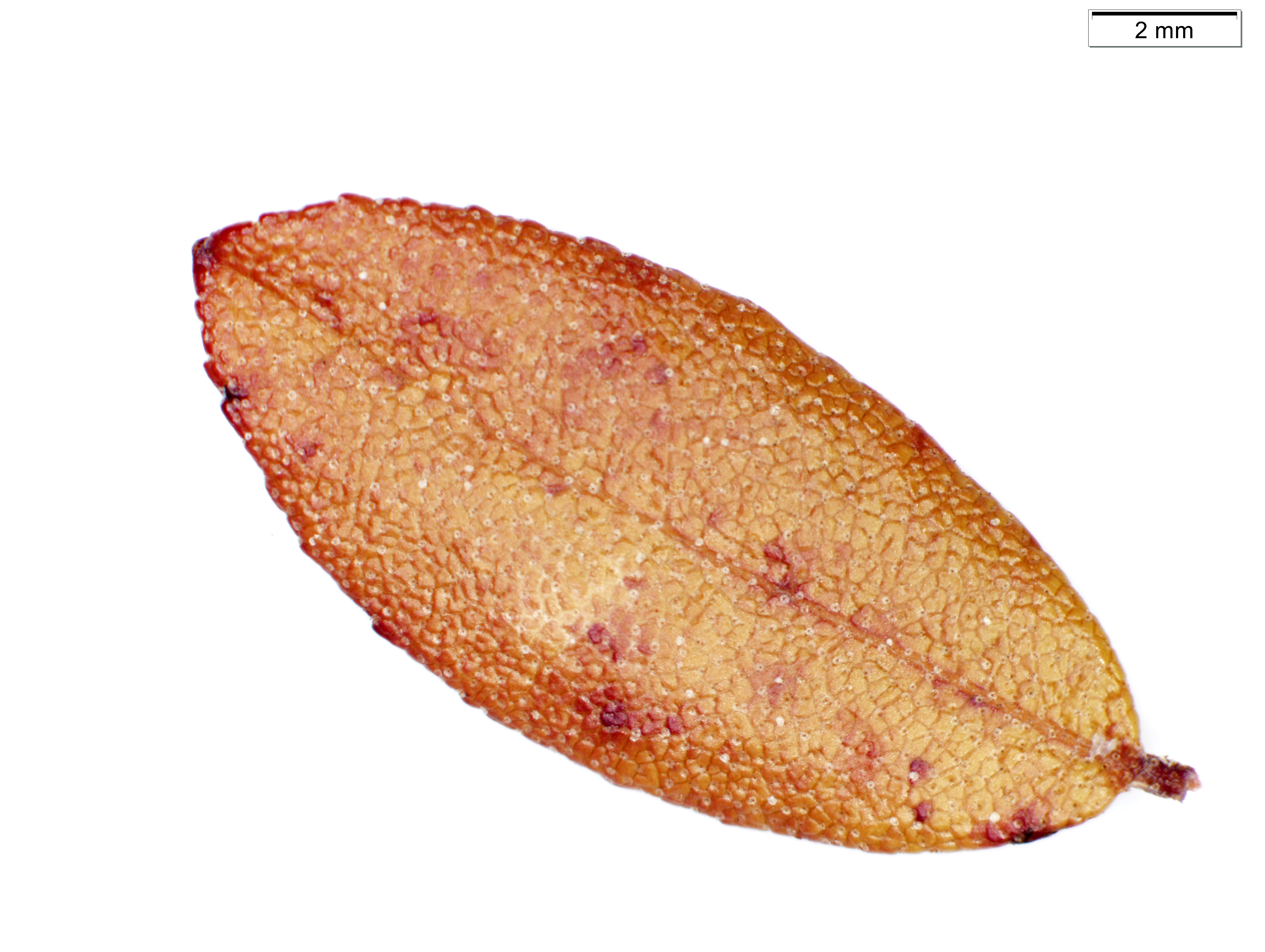 |
Leaf of terrestrial bog shrub Chamaedaphne calyculata. Dateable. |
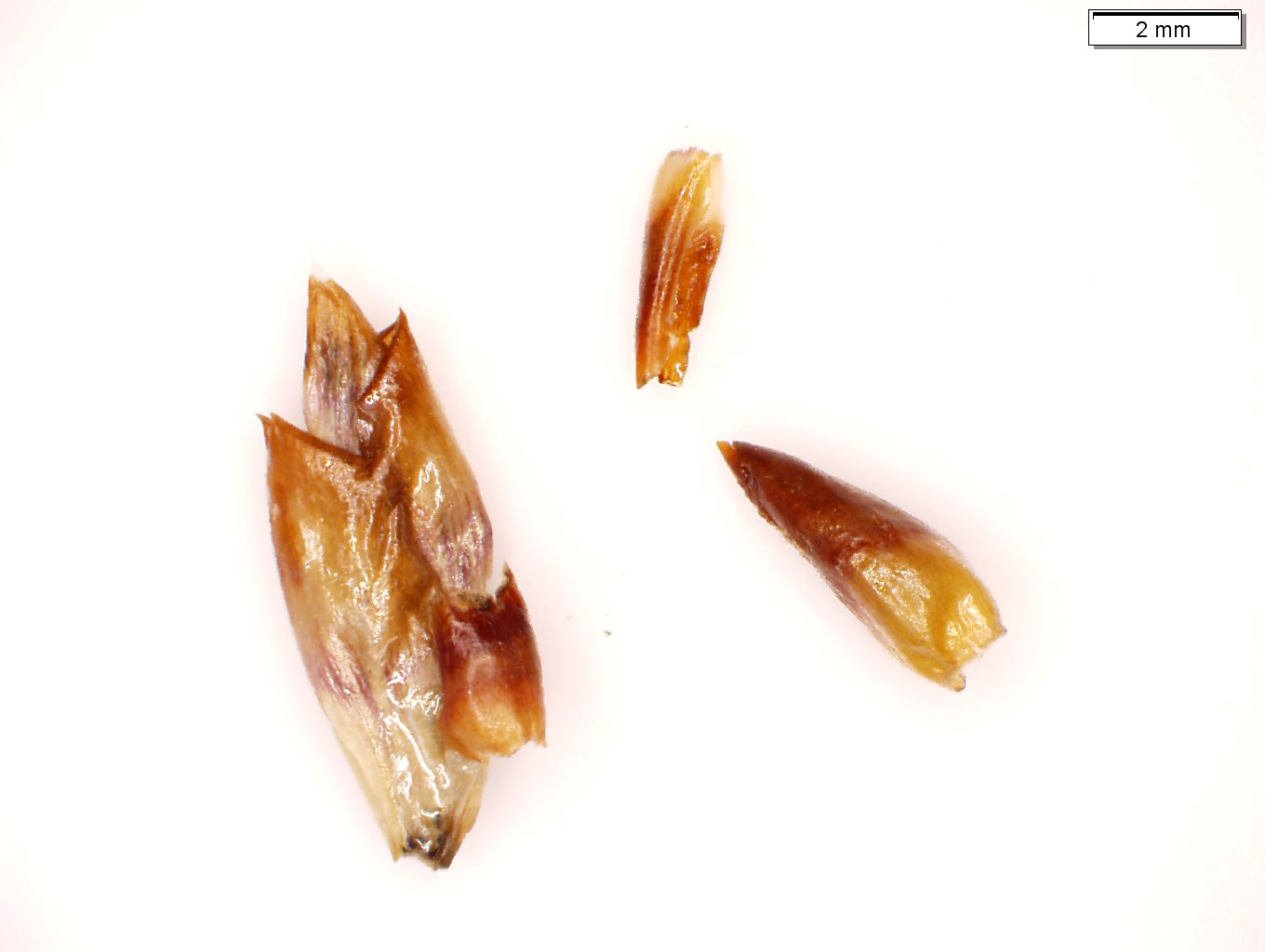 |
Bud scales of terrestrial tree Populus tremuloides. Dateable. |
Charcoal (from
grassland regions)
Grassland vegetation fixes only atmospheric CO2.
Grassland vegetation has a short terrestrial residence time; it is without
the in-built age present in long-lived, slow-decomposing tree species that may
remain on the landscape for significant lengths of time (Oswald et al 2005;
Grimm 2011).
Emergent-aquatic plant
macrofossils
Emergent macrophytes have a strong
history of providing good dates (Deevey et al 1954; Heikkinen et al 1977;
Hakansson 1982; Tornqvist et al 1992; Lowe et al 2004; Grimm 2011). Most
are unable to fix old carbon in the form of HCO
3- (Spence
and Maberly 1985; Sand-Jensen et al 1992; Maberly and Madsen 2002), and are
only able to utilize a very small portion of CO
2 absorbed
from the sediment and water. Yet there are some cases of emergent vegetation
returning dates that are too old (Damon et al 1964; Haynes et al 1966; Turney
et al 2000; Wasylikowa and Walanus 2004; Edwards et al 2011). Furthermore, Olsson
(1983) demonstrated that emergent and floating leaved vegetation have lower
14C
activities than terrestrial vegetation, though not as low as submerged
aquatics.
The physiological literature shows
that in some situations it is possible for old-carbon to be incorporated into
emergent vegetation if there is an old-CO
2 reservoir present. Amphibious
isoetids such as
Lobelia dortmanna
and
Juncus bulbosus (the seeds of which
can be easily confused with other
Juncus species)
acquire the majority of their CO
2 from the sediment via the roots (Wium-Anderson 1971; Winkel and Borum 2009). Recent
evidence shows that root uptake of CO
2 may not only be limited to
isoetids. In a hard water lake,
Sparganium
angustifolium utilized sediment CO
2 in amounts that
significantly affected productivity (Lucassen et al 2009).
Stratoides aloides is able to utilize HCO
3-
in significant amounts, the only known amphibious plant that can do so (Prins
and De Guia 1986). Additionally, new studies continue to suggest that emergent
plants may be able to fix aquatic CO
2 when submerged, albeit in
unknown quantities. Gas film layers on leaves of emergents like
Typha,
Phalaris, and
Phragmites
allow the utilization of aquatic CO
2 (Colmer and Pedersen 2008). Morphological
plasticity of leaves and roots permit other amphibious macrophytes to adjust CO
2
fixation depending on the environment (Mommer et al 2007; Rich et al
2012).
So, are the macrofossils of emergent
plants dateable? While there is a short list of clearly unsuitable taxa that
includes isoetids and
Stratoides aloides, it appears that most other
emergent macrophytes only utilize old carbon under very particular
circumstances and/or in very small or unknown quantities. When determining if an emergent macrofossil is suitable
for dating, it is extremely important to carefully consider the study
environment and the ecophysiological characteristics of the plant material. For example, if there is old CO
2 present in a lake, the suitability of
Sparganium fruits for dating is most likely dependent on whether
the lake of study is hard or soft water.
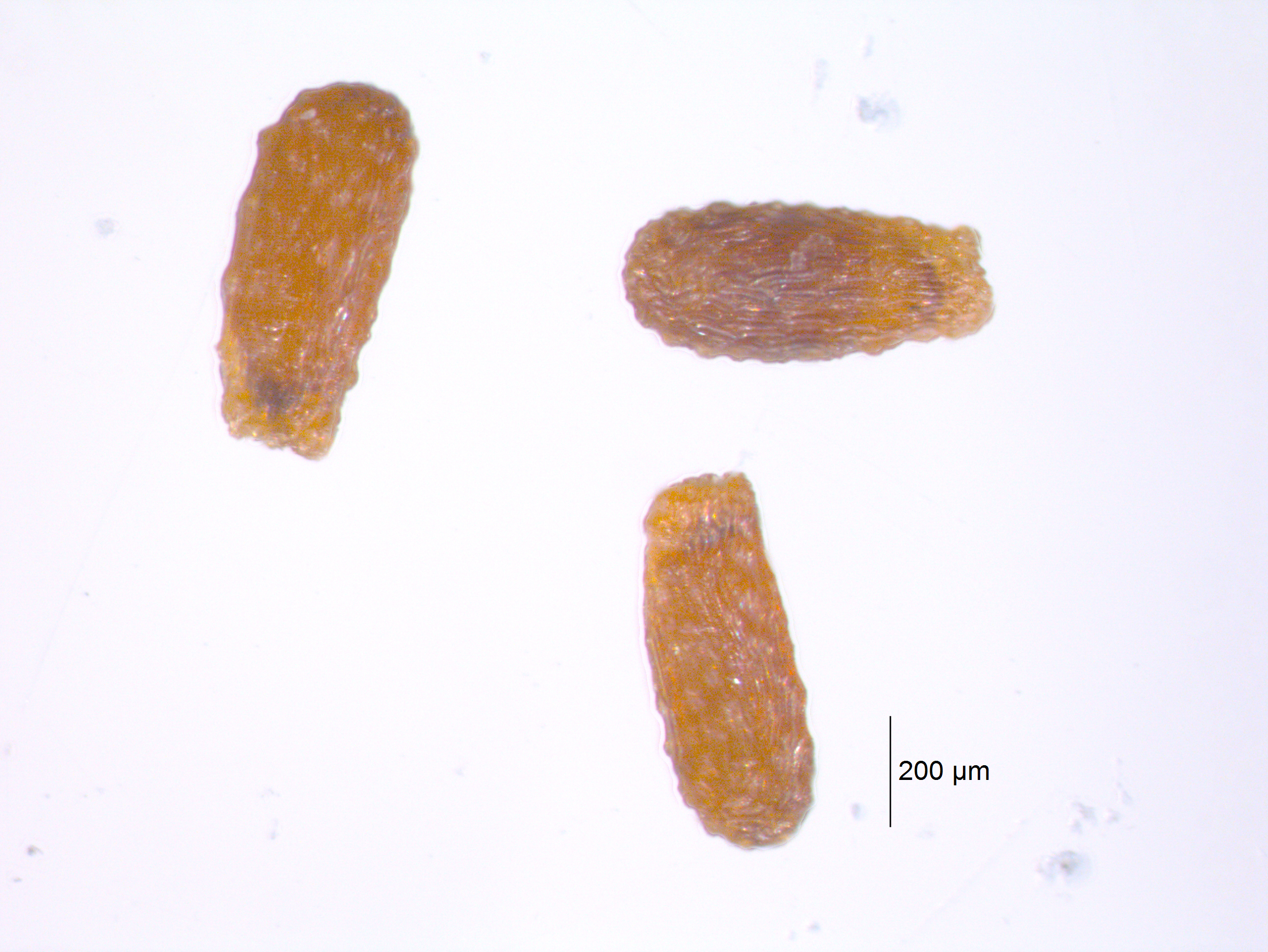 |
Seeds of emergent aquatic macrophyte Lobelia dortmanna (water lobelia). This species absorbs the majority of photosynthetic CO2 from the sediment (Wium-Anderson 1971). Do not date. |
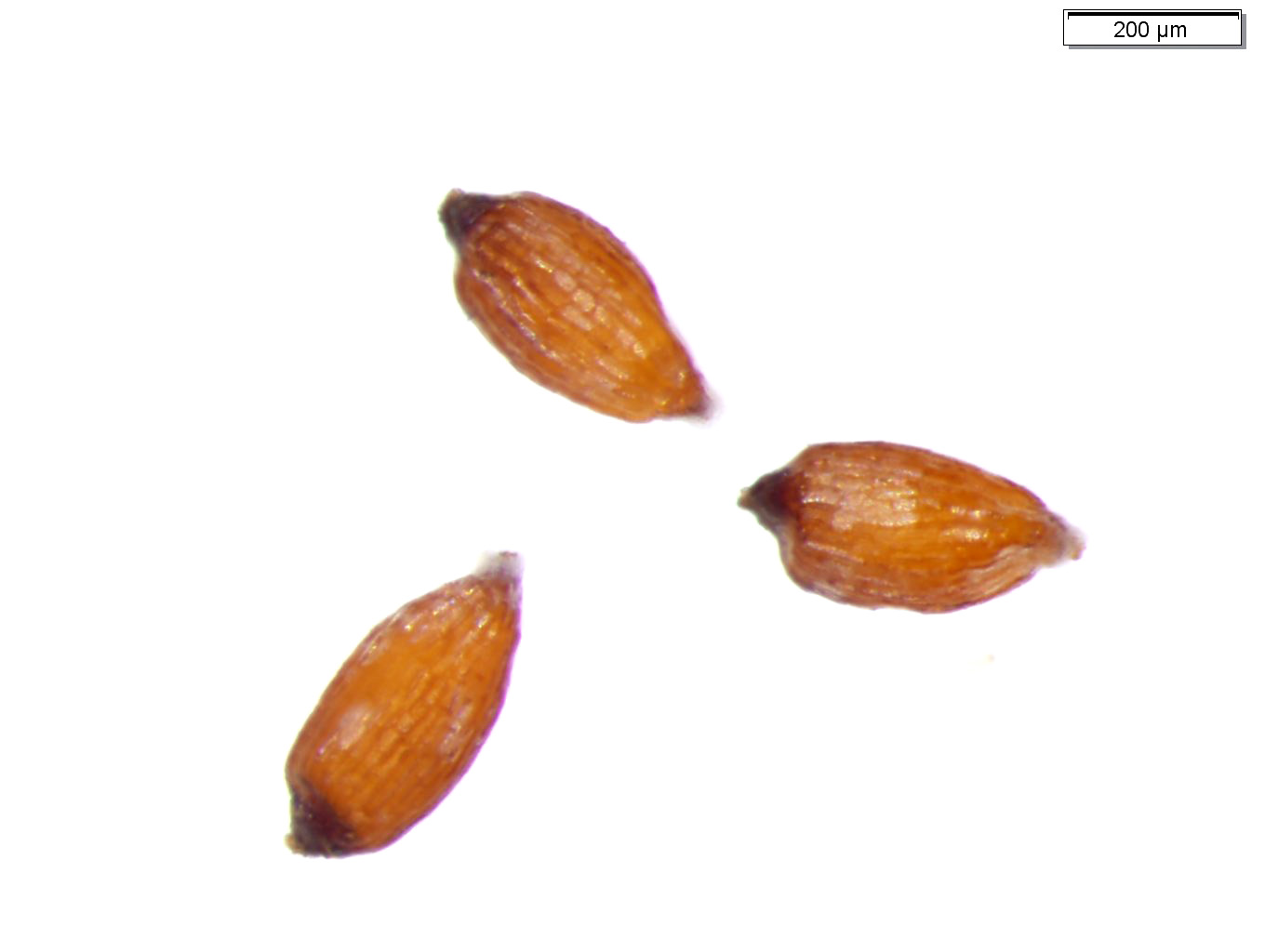 |
Seeds of emergent/submerged aquatic macrophyte Juncus pelocarpus (brown-fruited rush). This species of rush has both emergent and submerged forms, and a relative, Juncus bulbosus, fixes mostly sediment CO2 (Wetzel et al 1985). Date with caution. |
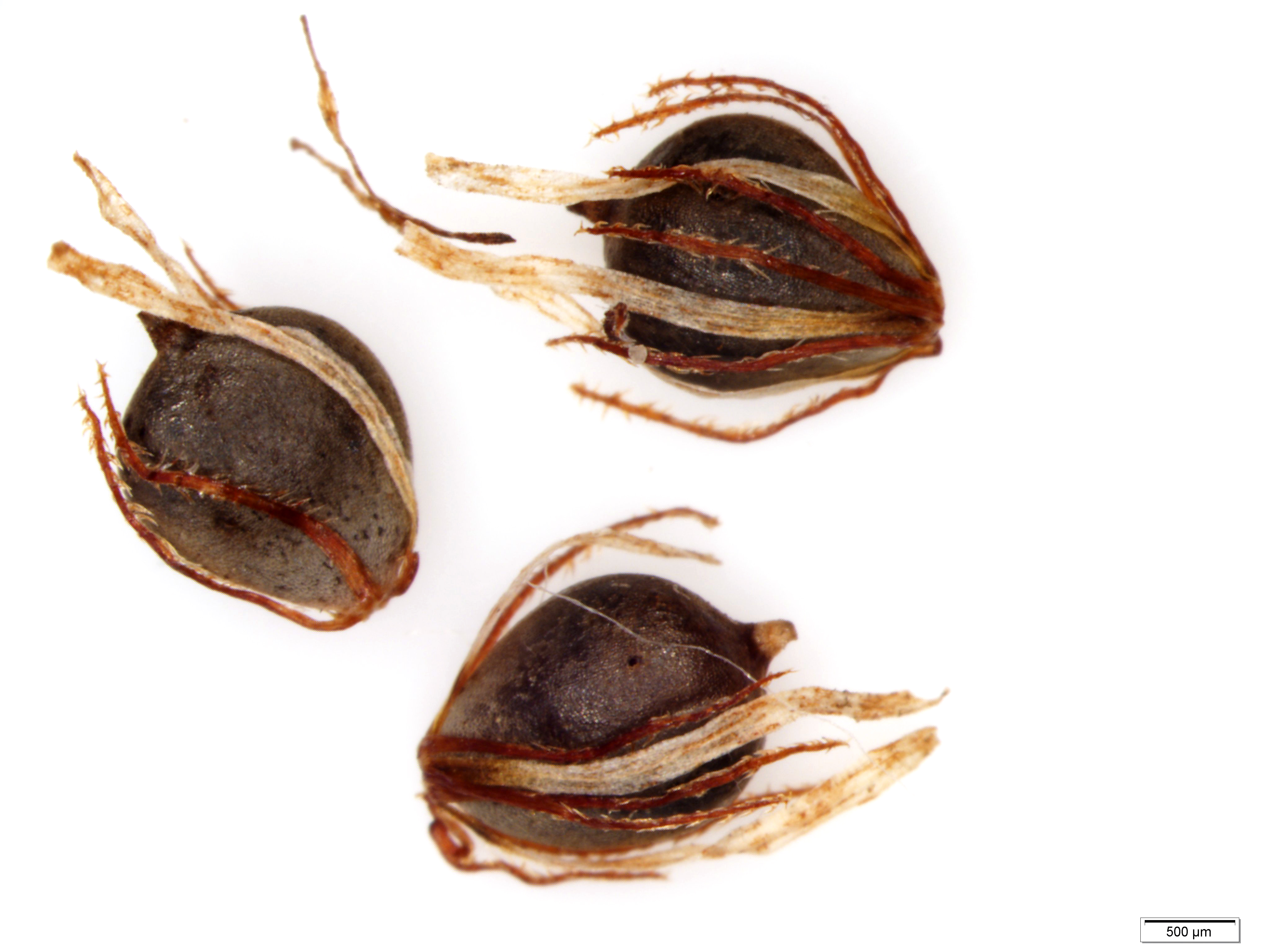 |
Seeds of emergent aquatic macrophyte Schoenoplectus acutus (hard stem bulrush). A European member of this genus (Schoenoplectus tabernaemontani) internalizes large amounts of sediment CO2, but only fixes very small quantities (Singer et al 1994). Date with caution.
|
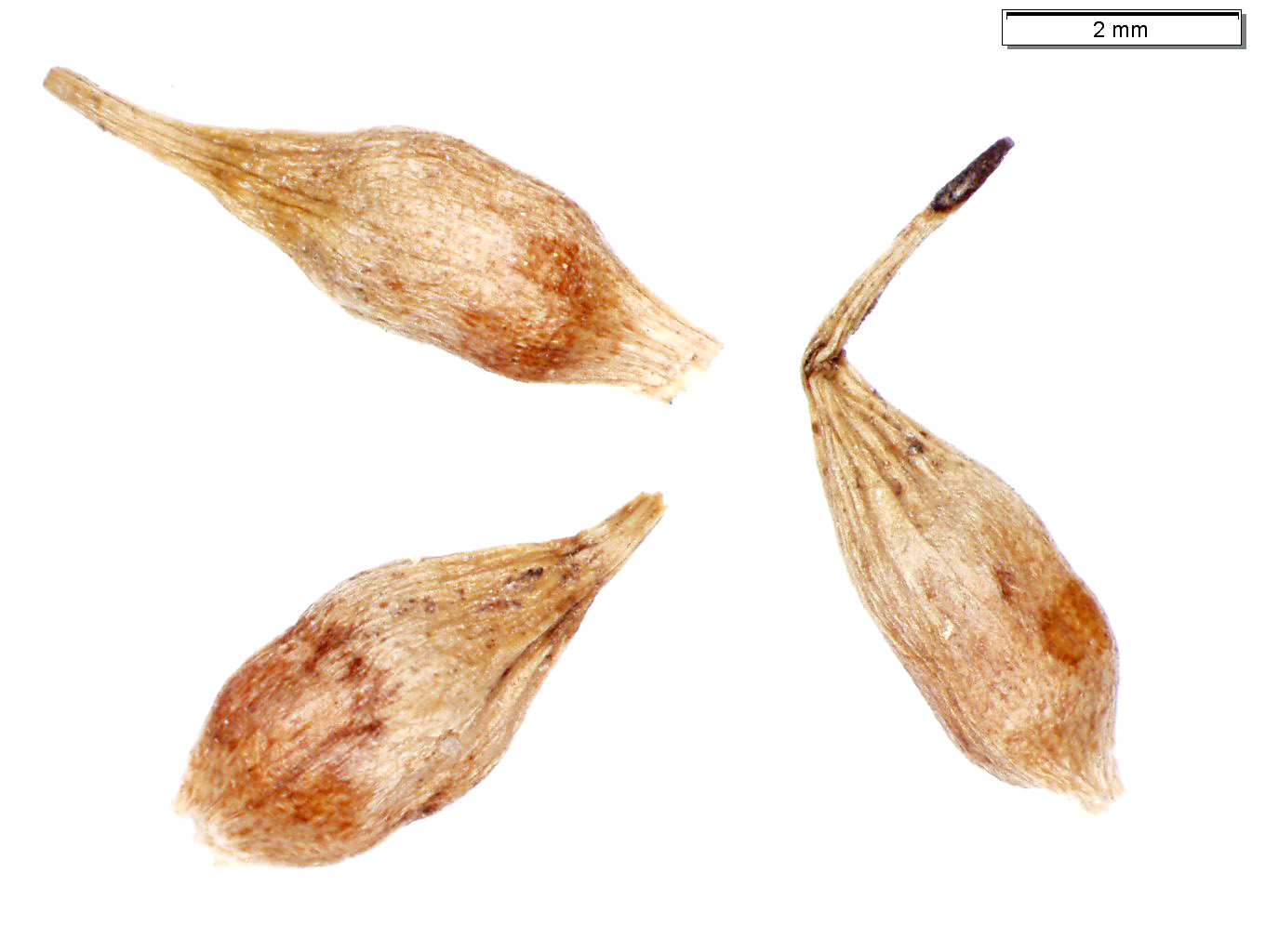 |
Fruits of emergent aquatic macrophyte Sparganium angustifolium (narrow leaf bur-reed). This species can utilize significant amounts of sediment CO2 in hard water conditions. Date with caution. |
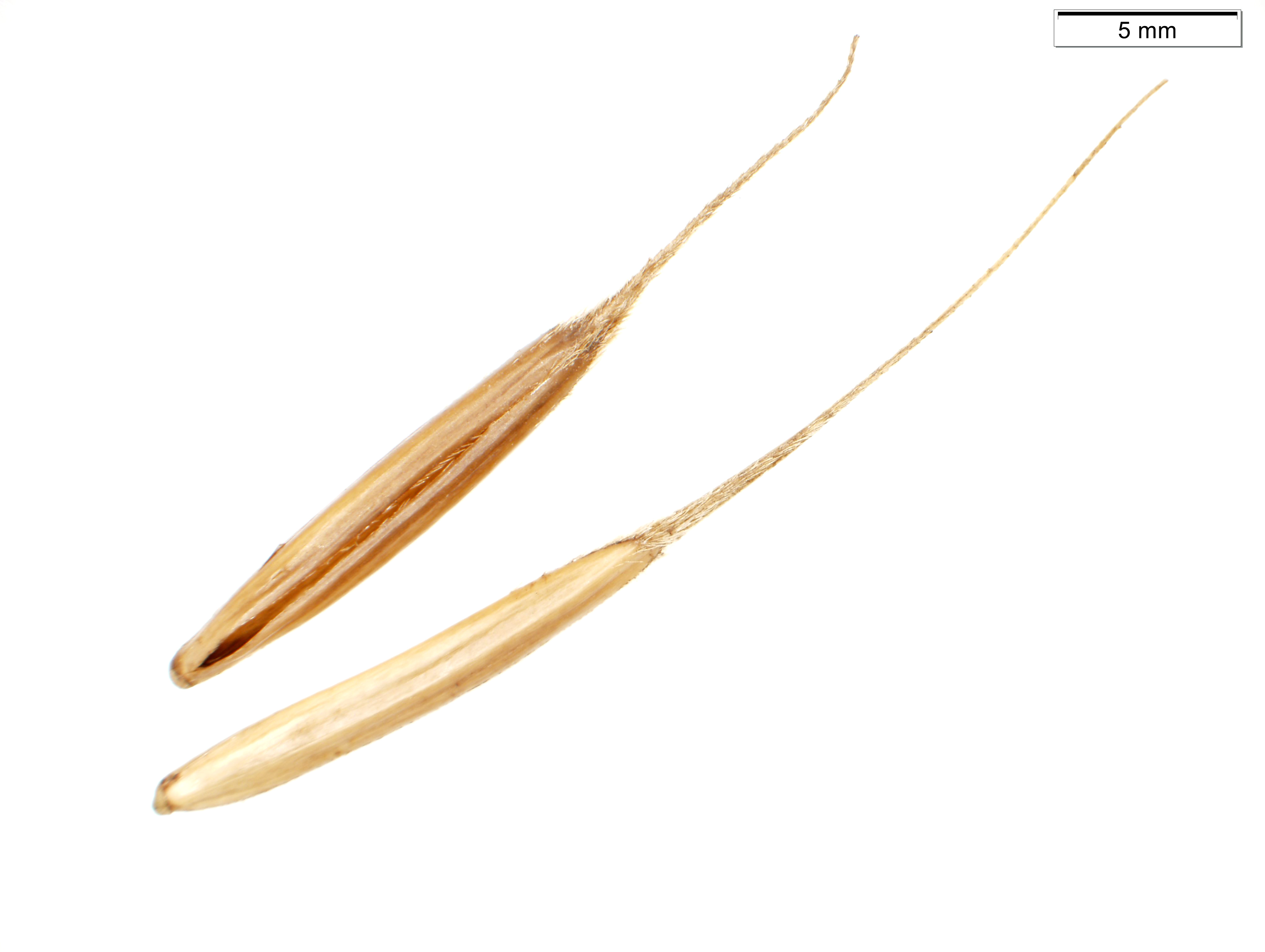 |
Spikelets of emergent aquatic macrophyte Zizania palustris (northern wild rice). Species of Asian rice, of the same tribe as Z. palustris, are adept at the uptake of sediment CO2 (Higuchi et al 1984). Date with caution. |
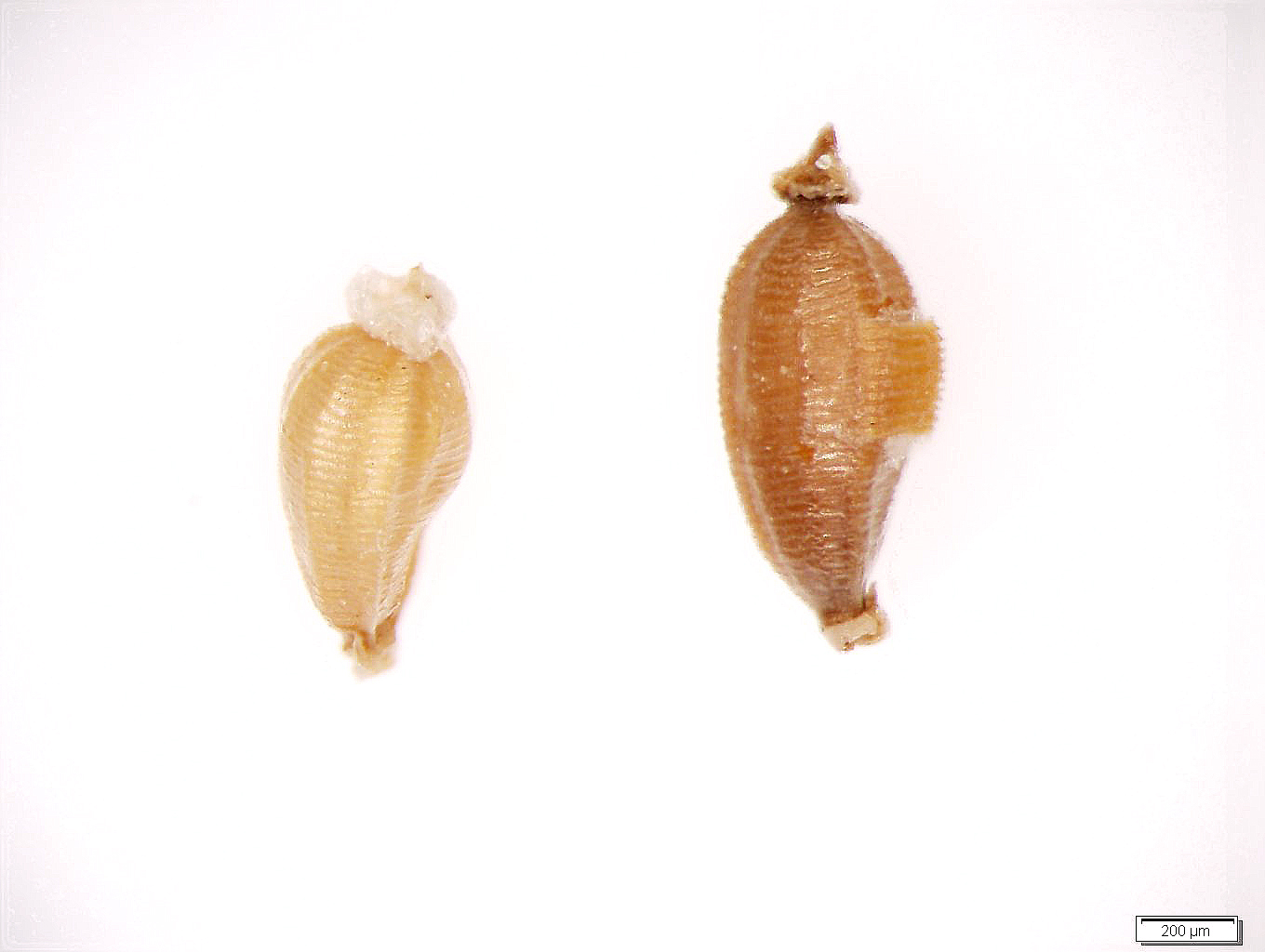 |
Seeds of amphibious macrophyte Eleocharis acicularis (needle spikerush). E. acicularis can grow under submerged or emergent conditions. A similar amphibious spikerush, Eleocharis vivipara uses aquatic CO2 when submerged (Ueno et al 1988). Date with caution. |
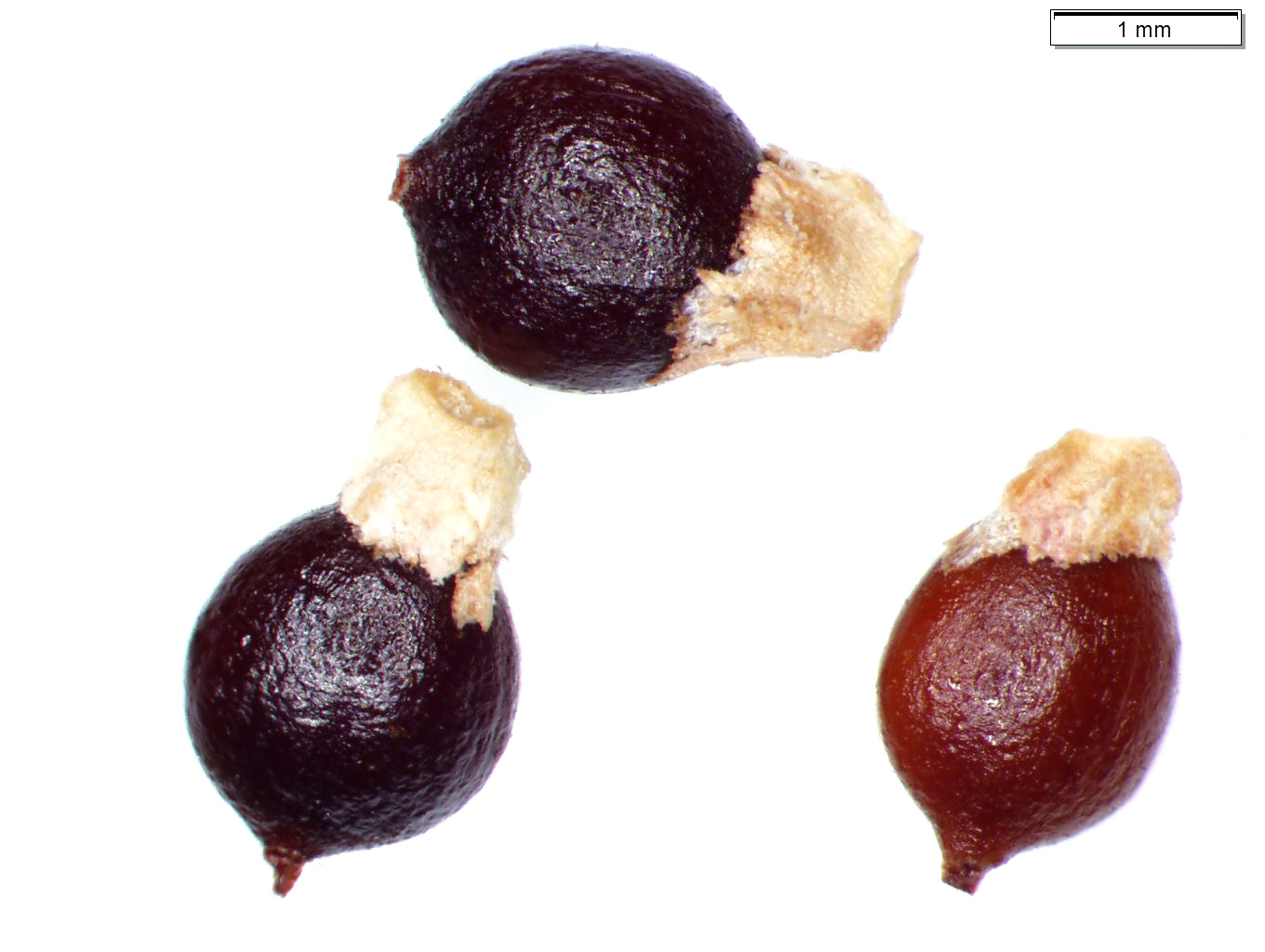 |
Seeds of emergent aquatic herb Polygonum amphibium (water smartweed). P. amphibium is able to produce both floating and aerial leaves based on water level conditions. Related species of the genus Rumex can produce submerged leaves that utilize aquatic CO2 (Mommer et al 2007). Date with caution. | |
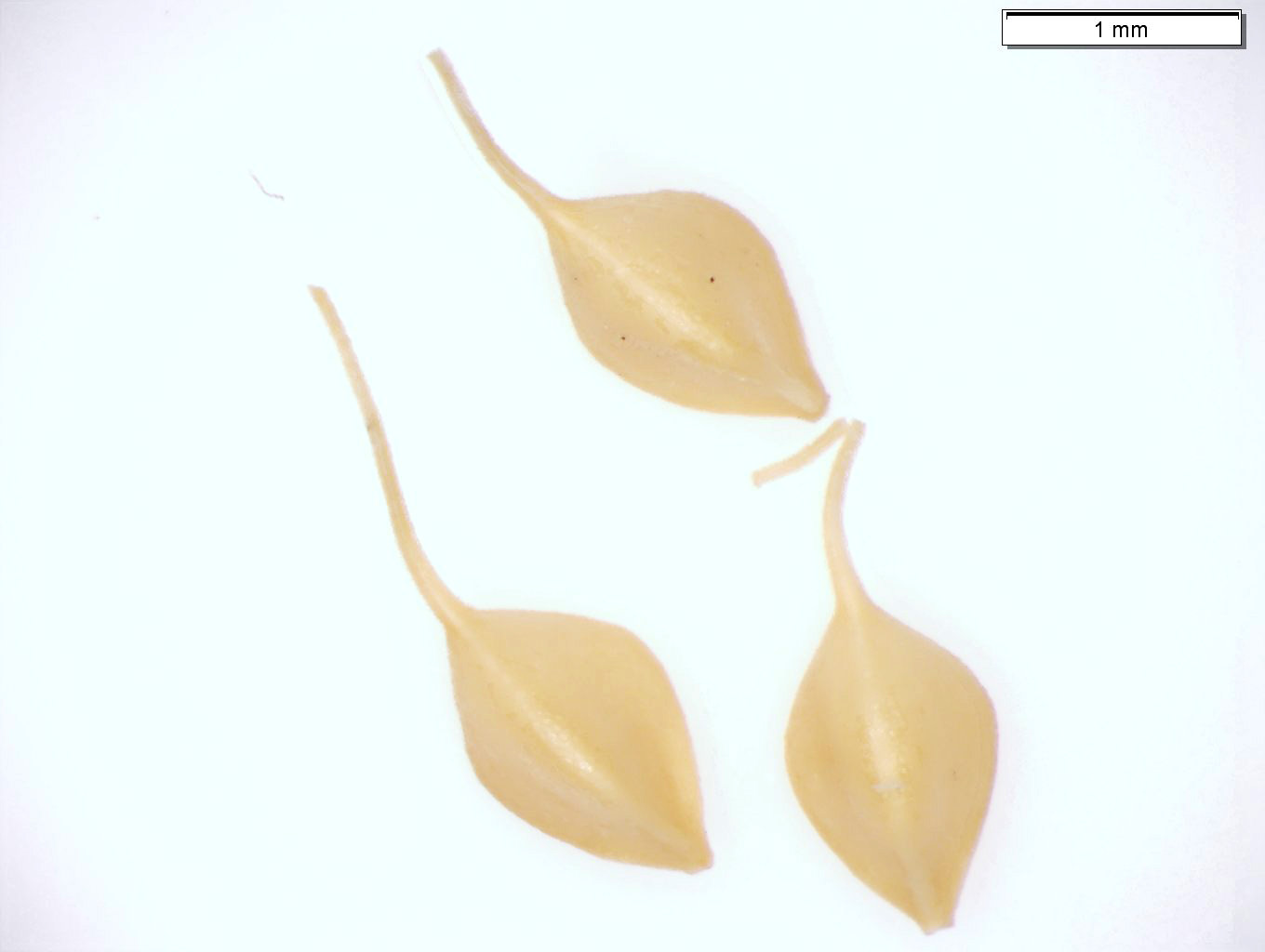 |
Seeds of emergent wetland plant Carex comosa (bristly sedge). There is flow of CO2 from root to shoot in some species of Carex, although it may not be fixed and appears to be respiratory (Koncalova et al 1988). Date with caution. |
Floating-leaved-aquatic plant macrofossils
Floating-leaved macrophytes
comprise a much smaller number of taxa than emergent macrophytes, with the
majority of research primarily involving nymphaeids (e.g. Nymphaea, Nuphar). Much
like emergent macrofossils, macrofossils of floating leaved macrophytes have
provided good dates in the past (Heikkinen et al 1977; Tornqvist et al 1992)
and are unable to fix old carbon in the form of HCO3-
(Spence and Maberly 1985; Sand-Jensen et al 1992; Maberly and Madsen 2002). This
is not the only similarity nymphaeids share with emergent macrophytes; the
suitability of nymphaeids for dating has also been questioned (Olsson 1983; Tornqvist
et al 1992; Olsson 2009). Physiological evidence suggests that Nuphar may take up CO2 from
the sediment, which is potentially problematic if the CO2 is derived
from an old-CO2 reservoir. In Nuphar
lutea, relatively large concentrations of CO2 move from the
rhizome to the upper parts of the plant where it is then fixed (Dacey and Klug
1982). The source of the CO2 is unknown and could be from either the
sediment or a product of respiration in the rhizome, the latter of which is a
product of atmospheric O2 (Dacey and Klug 1982). Because the
partial pressure of CO2 exceeds that of the partial pressure in the
rhizome, it is suggested that the CO2 is acquired from the sediment
(Dacey 1979). However, the amount of CO2 fixed through this pathway
is unknown, and may be insignificant in the context of radiocarbon dating. Like
with emergent macrofossils, one must evaluate the study environment carefully
to gauge the suitability of nymphaeid remains for dating. If there is an old CO2
reservoir available, proceed dating nymphaeids with caution.
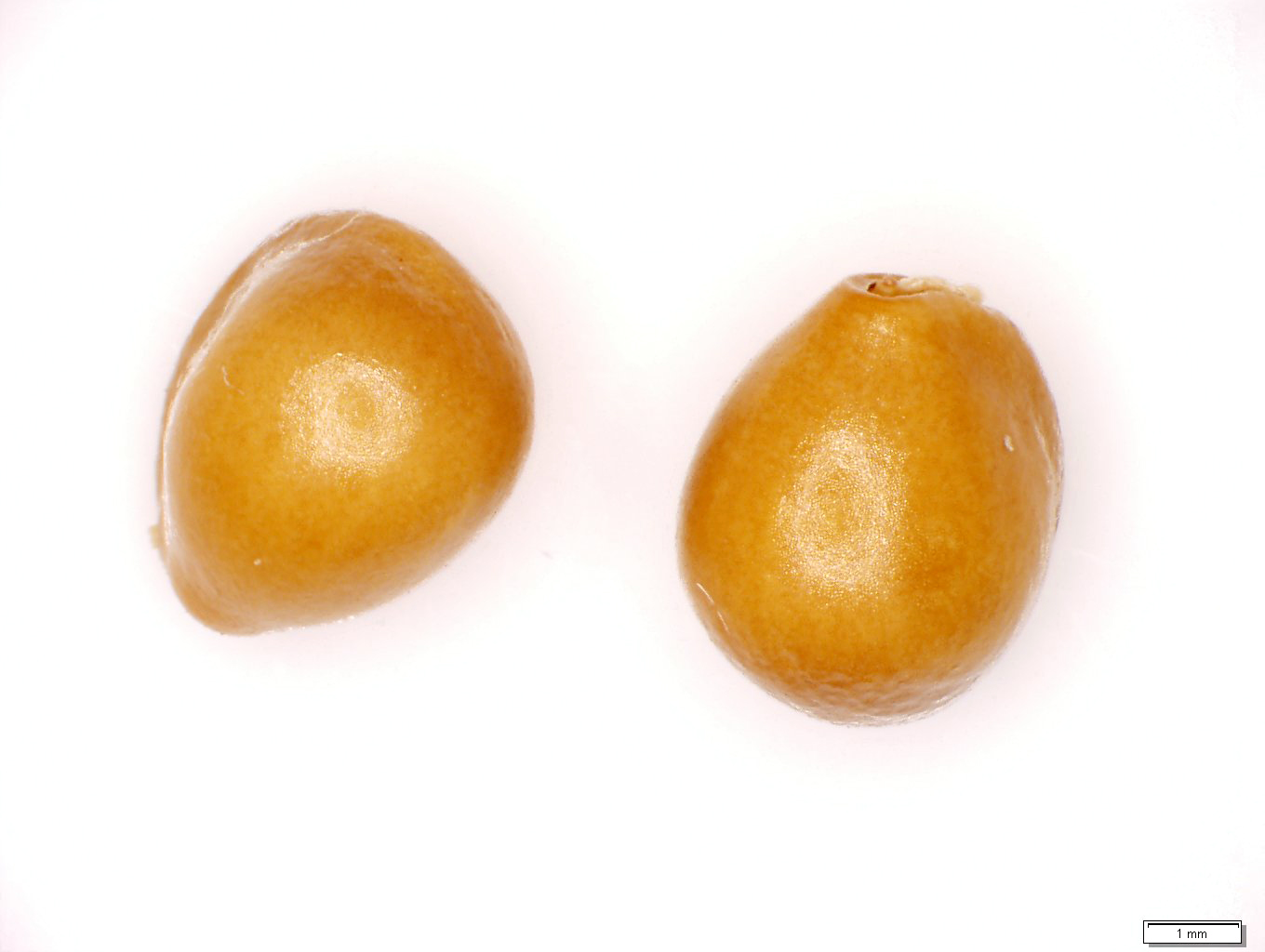 |
Seeds of floating-leaved aquatic macrophyte Nuphar lutea (yellow water lily, spatterdock). Date with caution. |
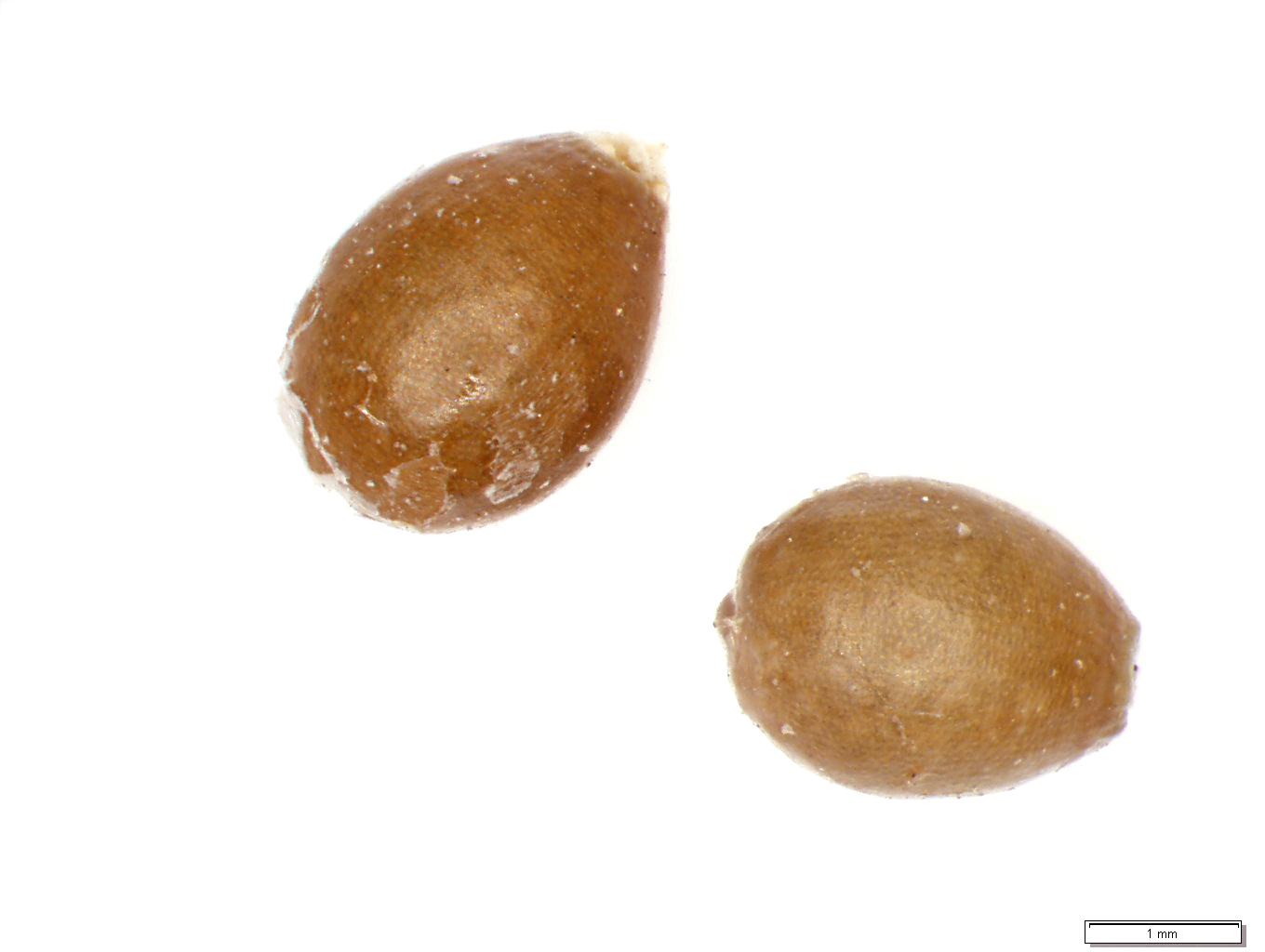 |
Seeds of floating-leaved macrophyte Nymphaea odorata (white water lily). Date with caution. |
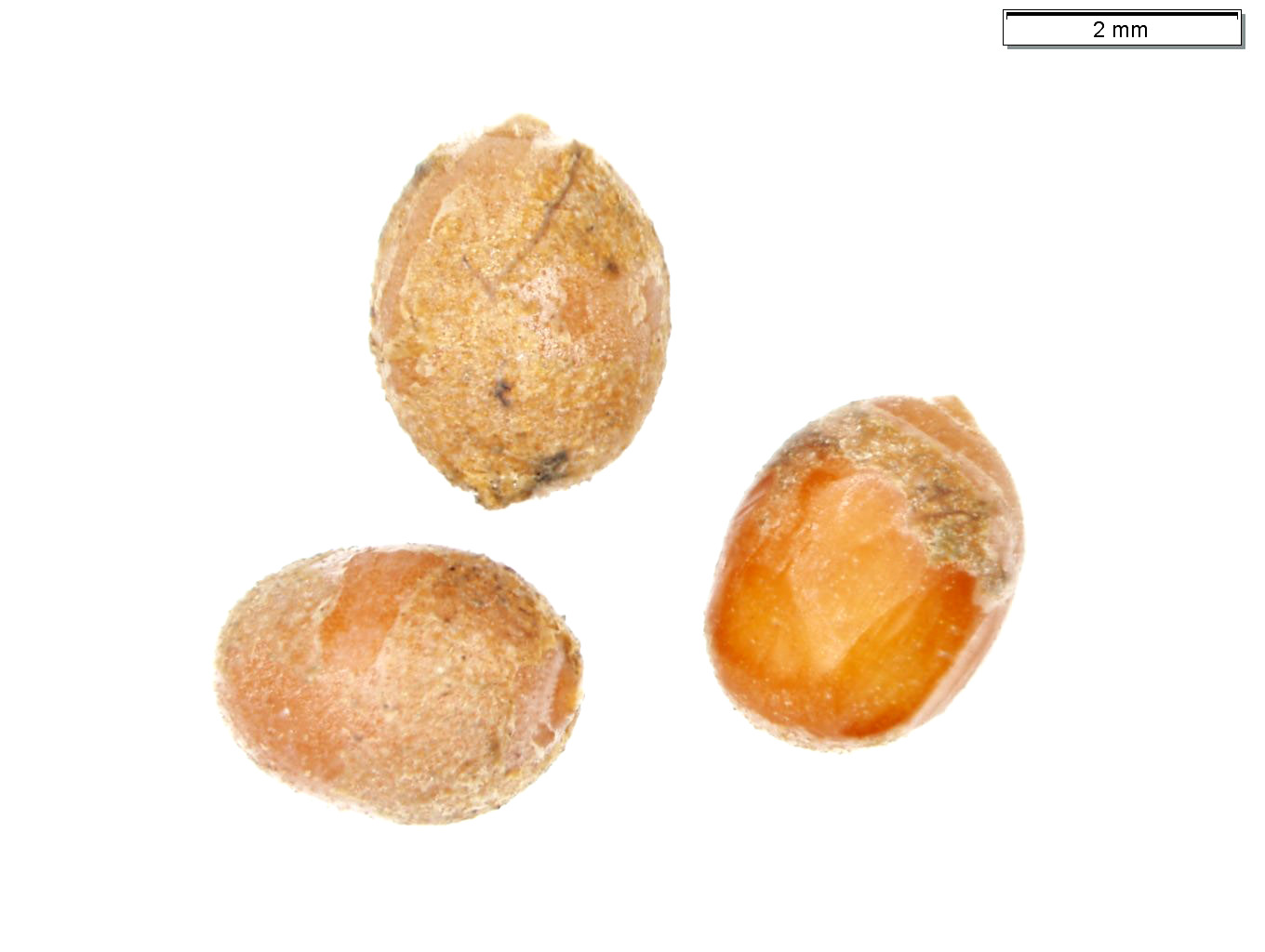 |
Seeds of floating-leaved macrophyte Brasenia schreberi (watershield). Date with caution. |
Wood charcoal
Woody plants fix only atmospheric CO2. However,
in some cases wood charcoal may provide inaccurate dates due to long
terrestrial residence times (Oswald et al 2005; Grimm 2011). Woody species
may take many years to decay and therefore can remain on the landscape for long
after atmospheric CO2 was originally fixed. Interpret dates from
wood charcoal with care.
Wood fragments
Like wood charcoal, wood may experience long terrestrial
residence times and provide inaccurate dates despite use of atmospheric CO2
(Oswald et al 2005; Grimm 2011). Furthermore, old carbon enriched material
may adhere to the rough surface texture of wood if the sample is not processes
correctly (Oswald et al 2005). For the best dates of woody material, look for
identifiable twigs of lakeshore species to ensure near-site deposition.
Submerged-aquatic
plant macrofossils
It is well
documented that macrofossils from submerged macrophytes and aquatic mosses return
radiocarbon dates that are spuriously old (Deevey et al 1954; Hakkanson 1979;
Olsson 1983; Birks 2002). Most submerged aquatic macrophytes are able to take
up aquatic HCO3- derived from carbonate bedrock that
results in radiocarbon dates many thousands of years too old (Spence and
Maberly 1985; Sand-Jensen et al 1992; Maberly and Madsen 2002). Aquatic
mosses and some submerged macrophytes do not utilize HCO3-;
rather they fix CO2 from the sediment (e.g. isoetids) and/or water
(e.g. Vallisneria americana), which
may still carry an old CO2 reservoir effect (Sand-Jensen et al
1992; Maberly and Madsen 2002). In very well mixed, soft water lakes, it may
be possible to date both submerged macrophytes and aquatic mosses (Hakansson
1979; Miller et al 1999; Oswald et al 2005). However, complete absence of a
reservoir effect is rare, and dating macrofossils of submerged
macrophytes and aquatic mosses is generally unadvisable (Olsson 2009).
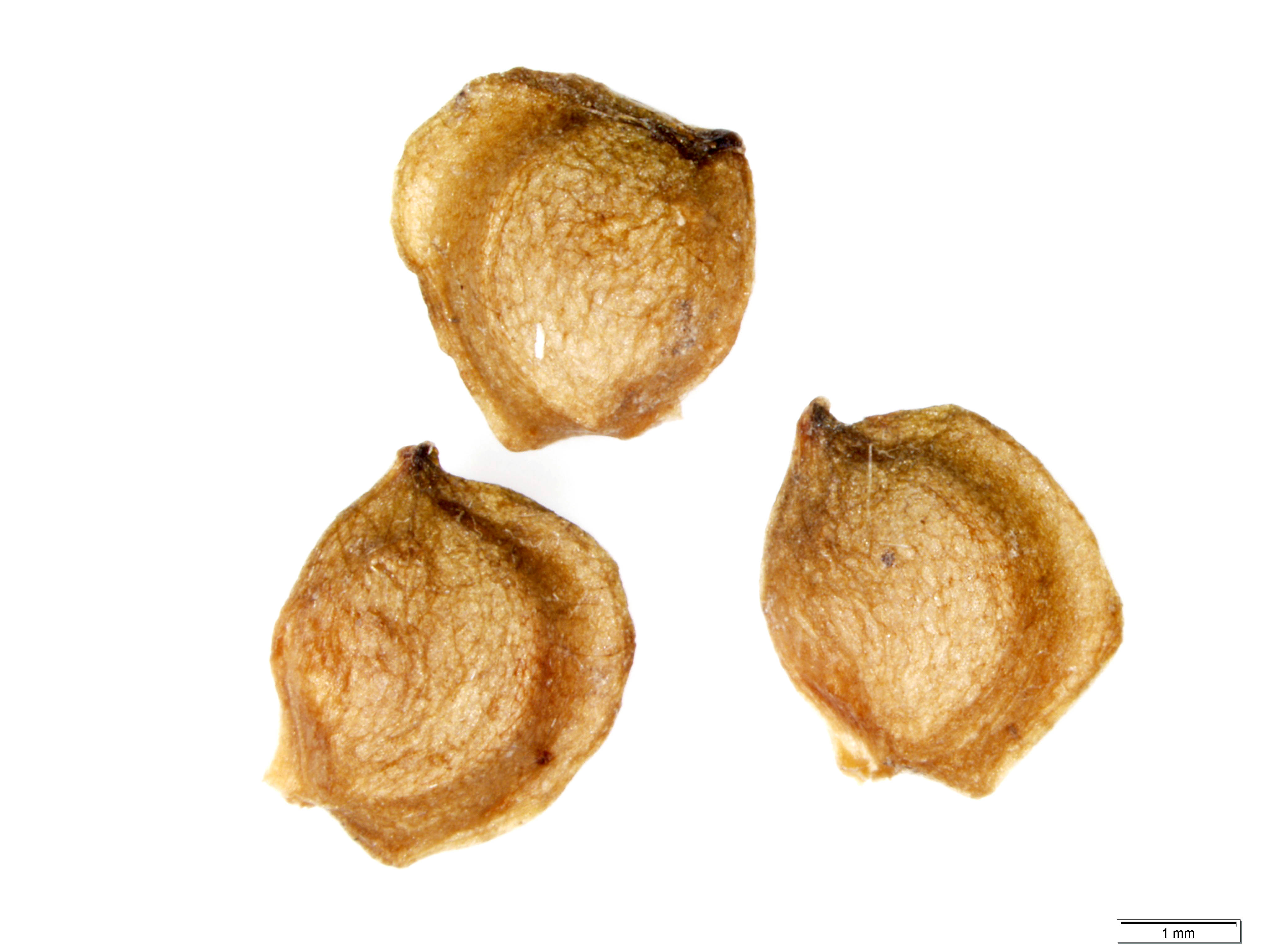 |
Seeds of submerged aquatic macrophyte Potamogeton epihydrus (ribbonleaf pondweed). Do not date. |
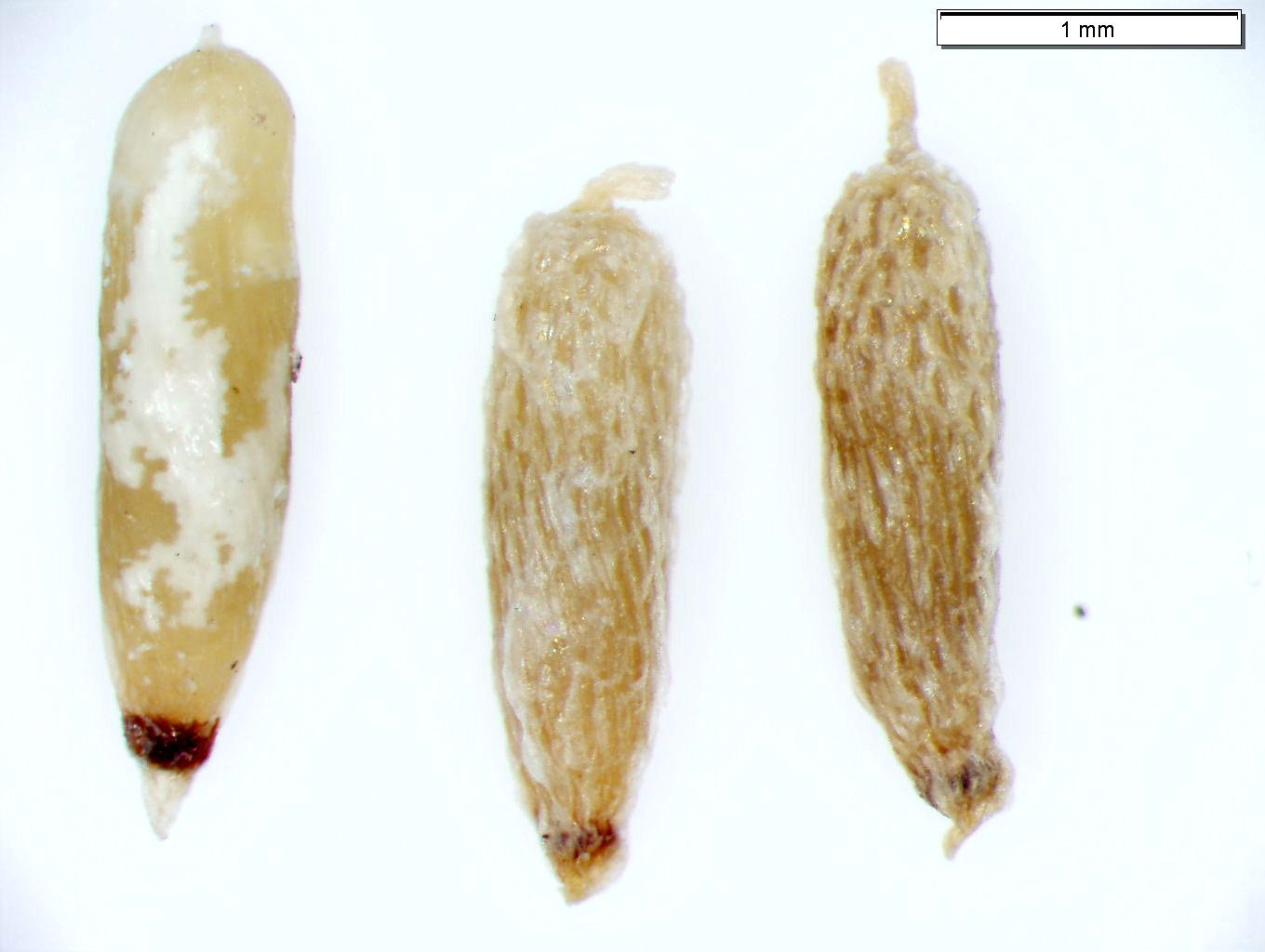 |
Seeds of submerged aquatic macrophyte Vallisneria americana (water celery). Do not date. |
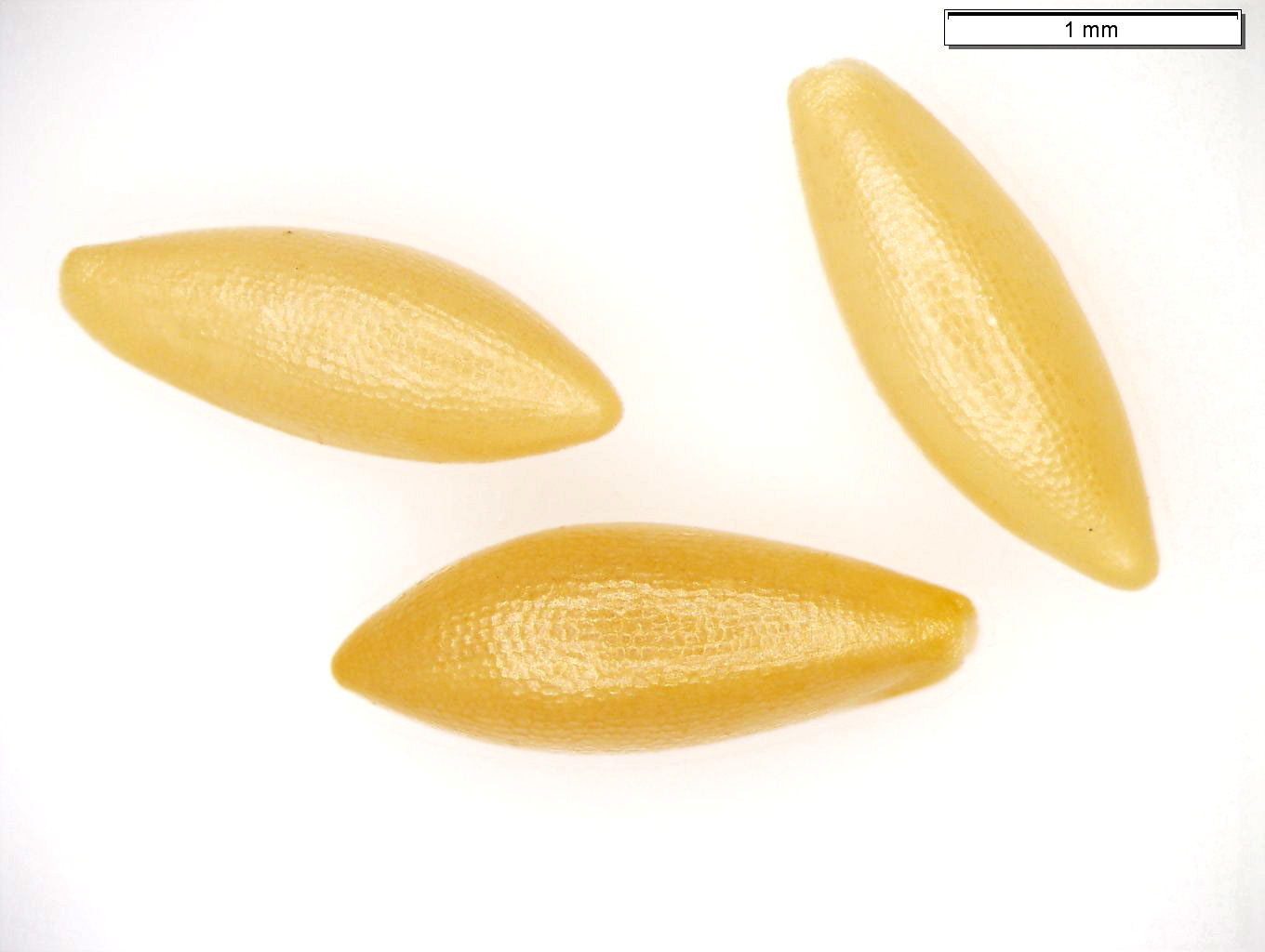 |
Seeds of submerged aquatic macrophyte Najas flexilis (nodding waternymph). Do not date. |
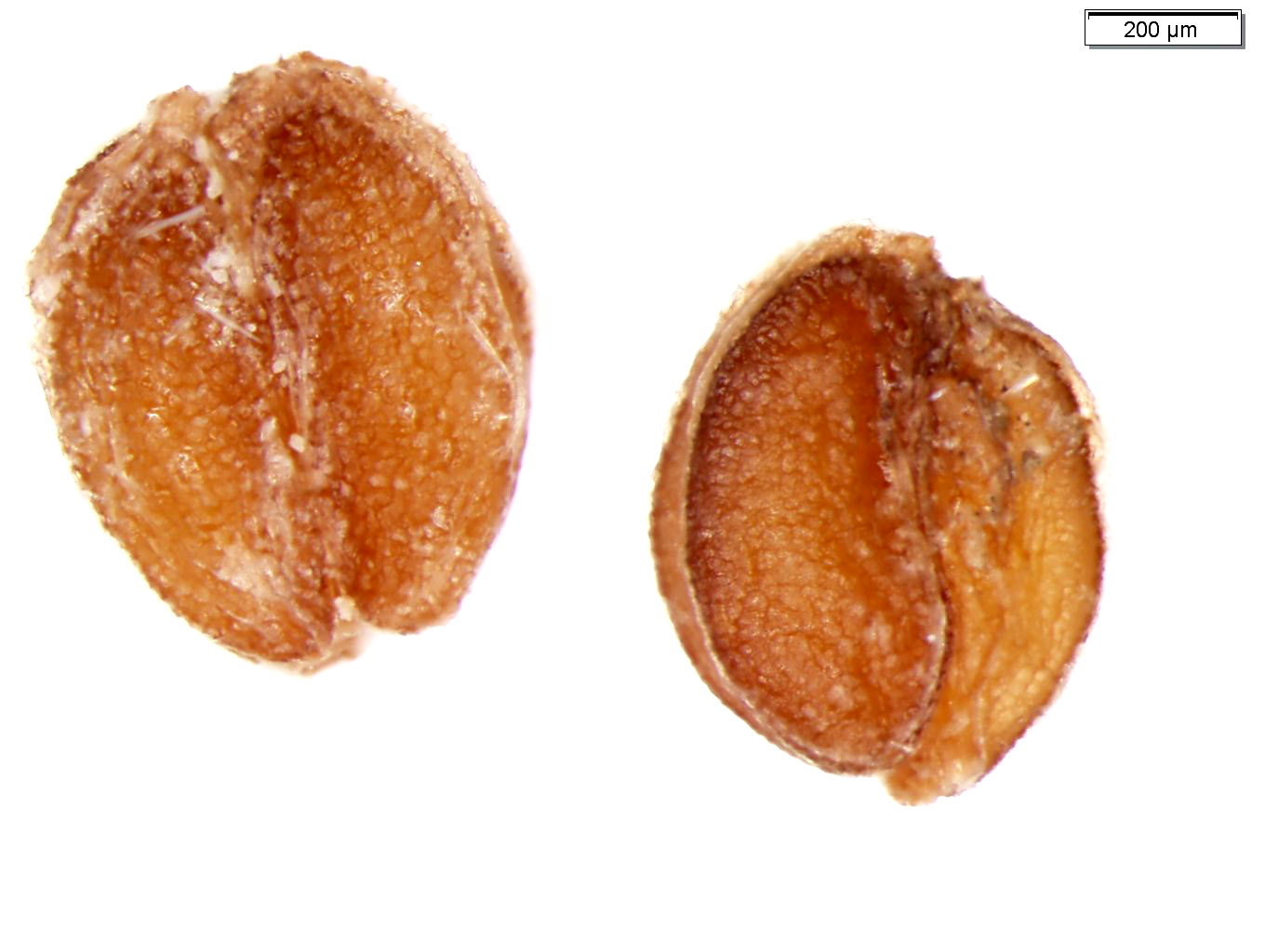 |
Seeds of submerged aquatic macrophyte Callitriche palustris (water starwort). Do not date. |
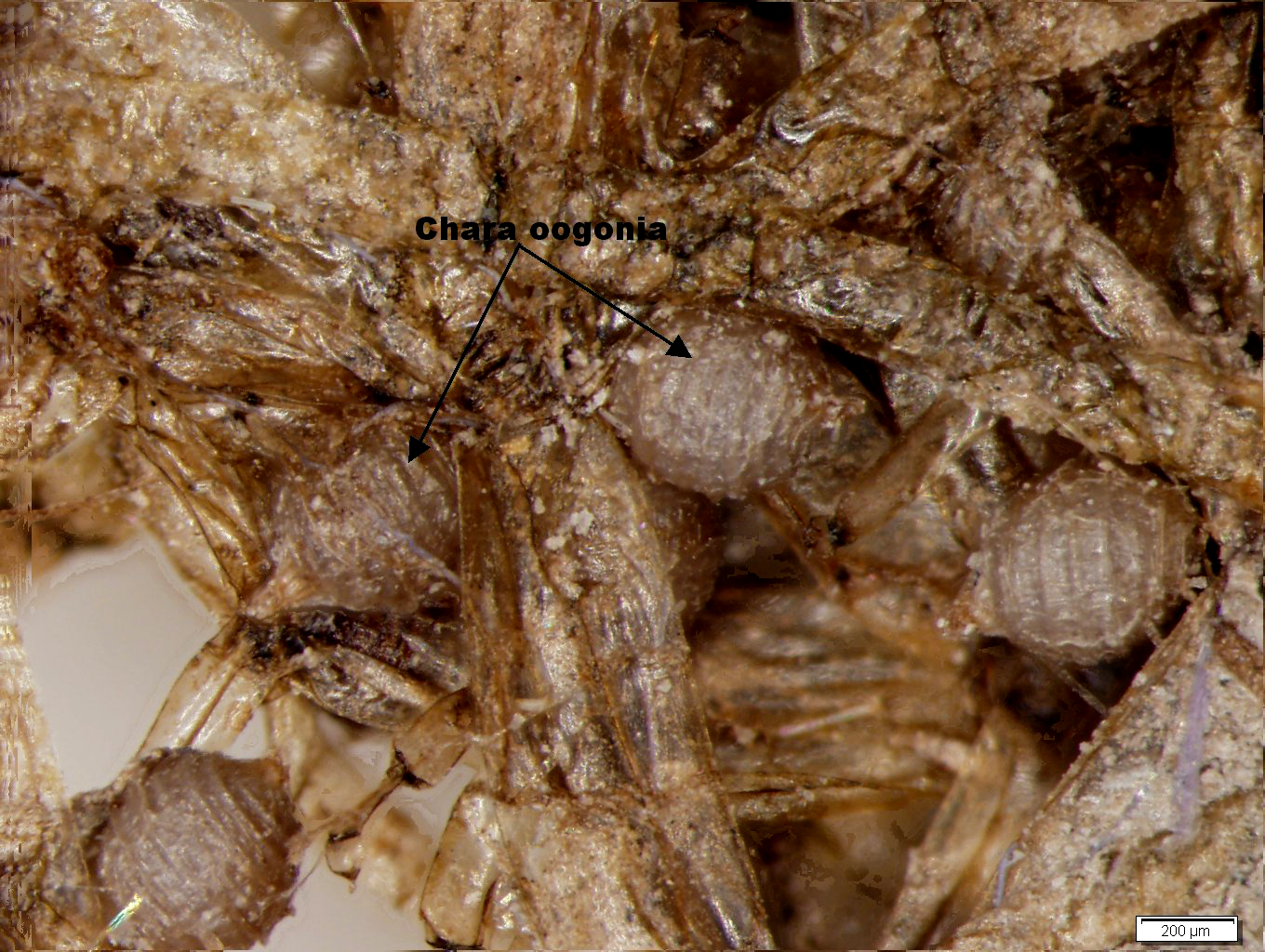 |
Oogonia of submerged aquatic macroalgae Chara sp. Do not date. | | |
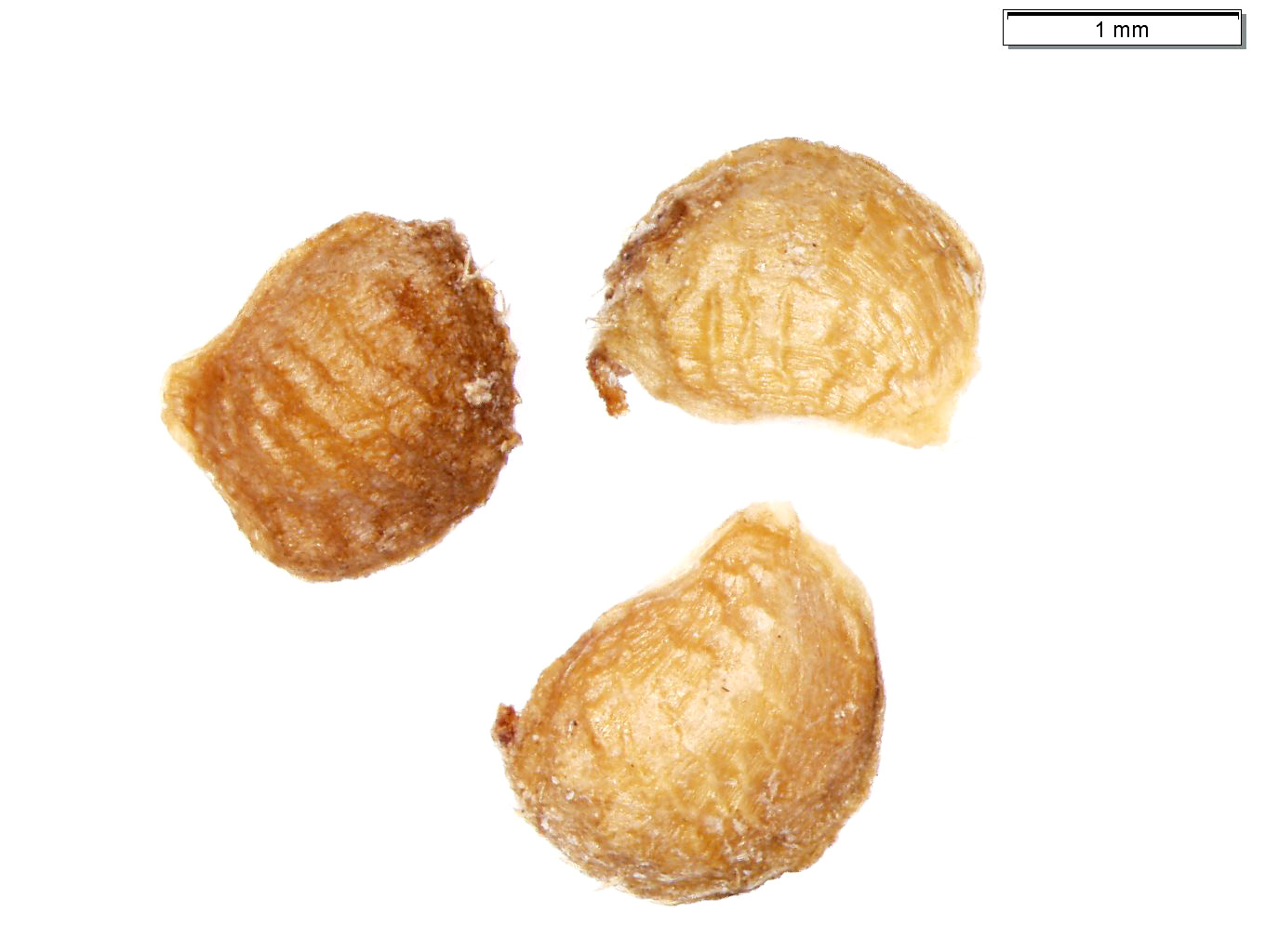 |
Seeds of submerged aquatic macrophyte Ranunculus aquatilus (white water crowfoot). Do not date. |
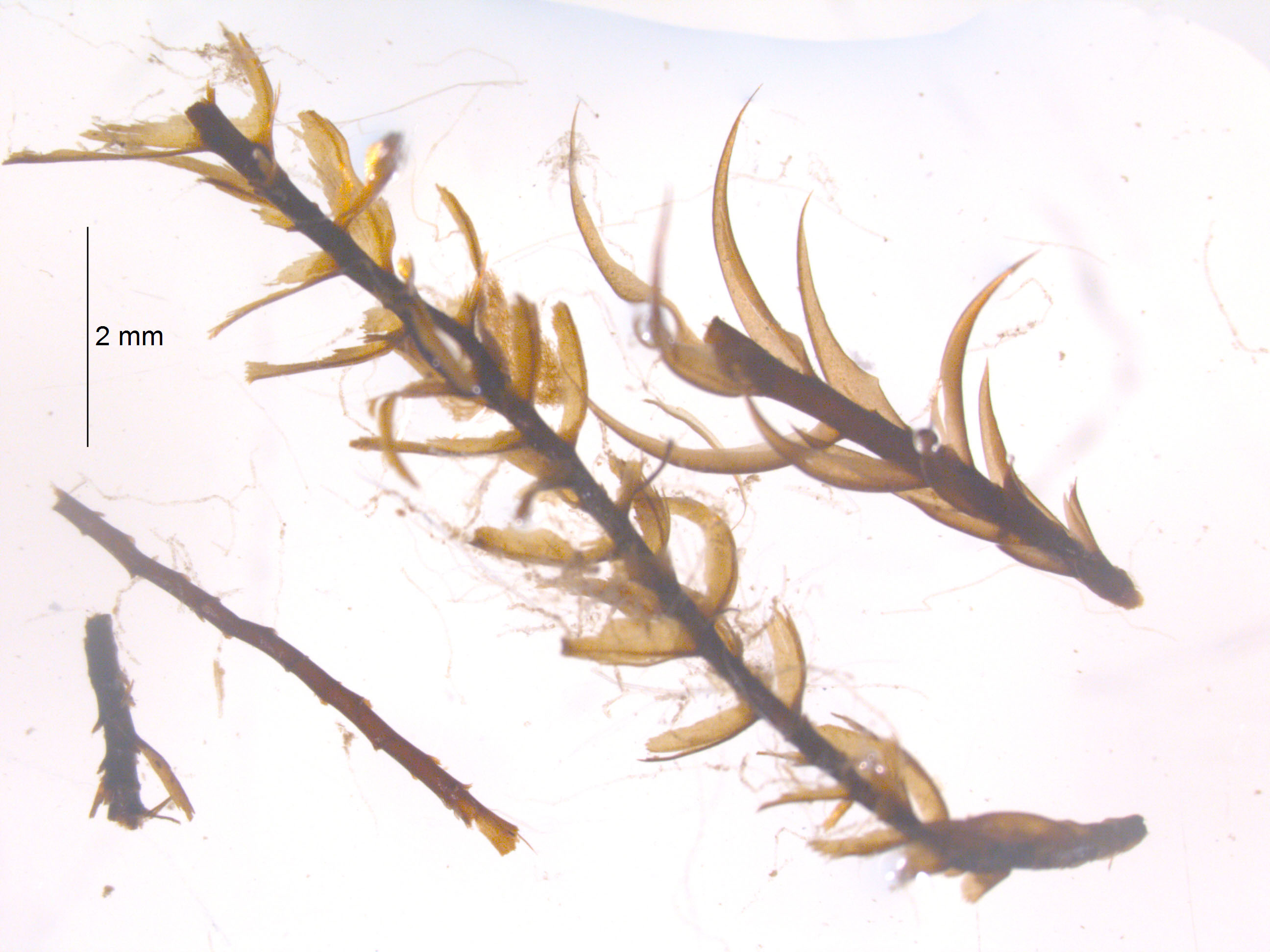 |
Aquatic moss fragments (unknown species). Do not date.
|
References cited
Birks, H.H., 2002, Plant macrofossils. In: Smol, J.P., Birks, H.J.B., Last, W.M. (Eds.), Tracking Environmental Change Using Lake Sediments, vol. 3: Terrestrial, Algal, and Siliceous Indicators. Kluwer Academic Publishers, Dordrecht, The Netherlands, p. 49-74.
Björck, S., and Wohlfarth, B., 2002, 14C
chronostratigraphic techniques in paleolimnology. In: Smol, J.P., Birks,
H.J.B., Last, W.M. (Eds.), Tracking Environmental Change Using Lake Sediments,
vol. 1: Basin Analysis, Coring, and Chronological Techniques. Kluwer Academic
Publishers, Dordrecht, The Netherlands, p. 205-245.
Colmer, T.D., and Pedersen, O., 2008, Underwater
photosynthesis and respiration in leaves of submerged wetland plants: gas films
improve CO2 and O2 exchange: New Phytologist, v. 177, p. 918-926.
Dacey, J.W.H., and Klug, M. J., 1982, Tracer studies of gas
circulation in Nuphar: 18O2
and 14CO2 transport: Plant Physiology, v. 56, p. 361-366.
Deevey, E.S., Gross, M.S., Hutchinson, G.E., and
Kraybill, H.L., 1954, The natural C14 contents of materials from
hard-water lakes: Geology, v. 40, p. 285-288.
Edwards, K.J., Schofield, J.E., Kirby, J.R., and Cook, G.T., 2011, Problematic but promising ponds? Palaeoenvironmental evidence from the
Norse Eastern Settlement of Greenland: Journal of Quaternary Science, v. 26, no. 8, p. 854-865.
Grimm, E.C., 2011, High-resolution age model based on AMS
radiocarbon ages for Kettle Lake, North Dakota, USA: Radiocarbon, v. 53, no. 1 , p. 39-53.
Hakansson S., 1979, Radiocarbon activity in submerged plants
from various south Swedish lakes. In: Berger, R., and Suess, H.E. (Eds.),
Radiocarbon Dating: Proceedings of the Ninth International Conference.
University of California Press, p. 433-443.
Hakansson S., 1982, University of Lund radiocarbon dates XV:
Radiocarbon, v. 24, no. 2, p. 194-213.
Heikkinen, A., and Aikaa, O., 1977, Geological survey of
Finland radiocarbon measurements VII: Radiocarbon, v. 19, no. 2, p. 263-279.
Higuchi, T., Yoda, K., and Tensho, K., 1984, Further evidence
for gaseous CO2 transport in relation to root uptake of CO2
in rice plant: Soil Science and Plant Nutrition v. 30, no. 2, p. 125-136.
Koncalova, H., Pokorny, J., Kvet, J., 1988, Root ventilation in Carex gracilis curt.: diffusion or mass flow?: Aquatic Botany, v. 30, p. 149-155.
Lowe, J.J., Walker, M.J.C., Scott, E.M., Harkness, D.D., Bryant, C.L., and Davies, S.M., 2004, A coherent high-precision radiocarbon chronology
for the Late-glacial sequence at Sluggan Bog, Co. Antrim, Northern Ireland:
Journal of Quaternary Science, v. 19, no. 2, p. 147-158.
Lucassen, E.C.H.E.T., Spierenburg, P., Fraaije, R.G.A.,
Smolders, A.J.P., and Roelofs, J.G.M., 2009, Alkalinity generation and sediment CO2
uptake influence establishment of Sparganium
angustifolium in softwater lakes: Freshwater Biology, v. 54, p. 2300-2314.
Maberly, S.C., and Madsen, T.V., 2002, Freshwater angiosperm
carbon concentrating mechanisms: processes and patterns: Functional Plant
Biology, v. 29, p. 393-405.
MacDonald, G.M., Beukens, R.P., Kieser, W.E., and Vitt, D.H.,
1987, Comparative radiocarbon dating of terrestrial plant macrofossils and
aquatic moss from the “ice-free corridor” of western Canada: Geology, v. 15, p. 837-840.
Miller, G.H., Mode, W.N., Wolfe, A.P., Sauer, P.E., Bennike, O., Forman, S.L., Short, S.K., and Stafford, T.K., 1999, Stratified interglacial
lacustrine sediments from Baffin Island, Arctic Canada: chronology and
paleoenvironmental implications: Quaternary Science Reviews v. 18, p. 789-810.
Mommer, L., Wolters-Arts, M., Andersen, C., Visser, E.J.W., and Pedersen, O., 2007, Submergence-induced leaf acclimation in terrestrial species
varying in flooding tolerance: New Phytologist, v. 176, p. 337-345.
Mook, W.G., 1980, Carbon-14 in hydrological cylces, in Fritz, P., and Fontes, J. Ch., eds., Handbook of environmental isotope geochemistry: Amsterdam, Elsevier, p. 49-74.
Olsson, I.U., 1983, Dating non-terrestrial materials. In:
Mook, W.G., and Waterbolk, H.T. (Eds.), Proceedings of the International
Symposium 14C and Archaeology. PACT v. 8, p. 277-294.
Olsson, I.U., 2009, Radiocarbon dating history: early days,
questions, and problems met: Radiocarbon, v. 51, no.1, p. 1-43.
Oswald, W.W., Anderson, P.M., Brown, T.A., Brubaker, L.B., Hu, F.S., Lozhkin, A.V., Tinner, W., and Kaltenrieder, P., 2005, Effects of sample mass
and macrofossil type on radiocarbon dating of arctic and boreal lake sediments:
The Holocene, v. 15, no. 5, p. 758-767.
Prins, H.B.A.,
and De Guia, M.B., 1986, Carbon source of the water soldier, Stratiotes aloides L.: Aquatic Botany,
v. 26, p. 225-234.
Sand-Jensen, K., Pederson, M.F., and Nielsen, S.L., 1992,
Photosynthetic use of inorganic carbon among primary and secondary water plants
in streams: Freshwater Biology, v. 27, p. 283-293.
Singer, A., Eshel, A., Agami, M., and Beer, S., 1994, The
contribution of aerenchymal CO2 to the photosynthesis of emergent
and submerged culms of Scirpus lacustris
and Cyperus papyrus: Aquatic Botany,
v. 49, p. 107-116.
Spence, D.H.N., and Maberly, S.C., 1985, Occurrence and
ecological importance of HCO3- use among aquatic higher
plants. In: Lucas, W.J., and Berry, J.A. (Eds.), Inorganic carbon uptake by
aquatic photosynthetic organisms, Proceedings of and International Workshop on
Bicarbonate Use in Photosynthesis, p. 125-143.
Turney, C.S.M., Coope, G.R., Harkness, D.D., Lowe, J.J., and Walker, M.J.C., 2000, Implications for the dating of Wisconsian (Weichselian)
late-glacial events of systematic radiocarbon age differences between
terrestrial plant macrofossils from a site in SW Ireland: Quaternary Research, v.
53, p. 114-121.
Ueno, O., Samejima, M., Muto, S., and Miyachi, S., 1988,
Photosynthetic characteristics of an amphibious plant, Eleocharis vivipara: expression of C4 and C3 modes
in contrasting environments: PNAS, v. 85, p. 6733-6737.
Wasylikowa, K., and Walanus, A., 2004, Timing of aquatic and
marsh-plant successions in different parts of Lake Zeribar, Iran, during the
Late Glacial and Holocene: Acta Palaeobotanica, v. 44, no. 2, p. 129-140.
Winkel, A., and Borum, J., 2009, Use of sediment CO2
by submersed rooted plants: Annals of Botany, v. 103, p. 1015-1023.
Wium-Andersen, S., 1971, Photosynthetic uptake of free CO2
by the roots of Lobelia dortmanna:
Plant Physiology, v. 25, p. 245-248.
Wohlfarth, B., Possnert, G., Skog, G., and Holmquist, B., 1998, Pitfalls in the AMS radiocarbon-dating of terrestrial macrofossils: Journal of Quaternary Science, v. 13, p. 137-145.